The present and the prospect of bioengineering cornea
Introduction
Corneal disease affects more than 10 million people worldwide and, after cataracts, is the second leading cause of blindness (1). Corneal transplantation is currently the standard treatment for restoring vision in the most severe cases (2). Although corneas are the most transplanted tissues, eye bank programs cannot fulfill the demand for transplants; therefore, long waiting lists are still a limiting factor (3). With the notable exception of North America, however, in most of the world the demand for high-quality donors exceeds the supply (4). The aging populations, the increase in corneal refractive surgeries and infectious diseases will further aggravate the shortage of donor problem. Therefore, there is an enormous demand for an alternative to donor corneal graft transplantation.
Synthetic keratoprosthesis (Kpro) and bioengineering corneas
Several attempts have been made in searching for suitable corneal substitutes. Such devices must fulfill the natural functions of the cornea: maintain transparency; having an adequate refractive index; protecting the inner ocular structures from external hazards, including pathogens and ultraviolet (UV) light; having proper mechanical properties to tolerate the intraocular pressure and allow diffusion of oxygen and nutrients (5). In addition, as biomaterials, they must be biocompatible, nontoxic, neither immunogenic nor mutagenic and integrate well with the recipients’ surrounding tissues and cells (6). There are two main substitute categories: synthetic Kpro and, our main interest, bioengineering corneas.
The Kpros were the first corneal substitute developed and was designed for patients suffering from severe diseases of the ocular surface, such as Stevens-Johnson syndrome (SJS) and ocular cicatricial pemphigoid (OCP), who are unable to maintain ocular surface function. Short-term visual recovery of Kpro (no matter the Boston Kpro, the osteo-odonto Kpro or the AlphaCor Kpro) is good but long-term prognosis is limited by various complications, including glaucoma, stromal melting, persistent epithelial defects and retroprosthetic membrane formation, requiring a high level of medical intervention, thus, reducing the long-term use of these devices (7). Compared with synthetic Kpros, tissue engineering corneal substitutes promise to overcome these challenges and deficiencies. Bioengineering corneas are constructed by naturally generating an extracellular matrix (ECM) component as the scaffold structure with or without corneal cells. It is well established that the scaffold structure directs the fate of cells, therefore, the fabrication of the correct scaffold structure components could produce an ideal corneal substitute, able to mimic the native corneal function.
Scaffold structure of bioengineering cornea
Direct in vivo implantation of corneal equivalent biomaterials without cells has been investigated to study the integration of scaffold structure with native corneal tissue. Biosynthetic corneas from cross-linked recombinant human collagen type III as scaffold structure were implanted in an anterior partial keratoplasty surgery in human patients, to enhance endogenous tissue regeneration. The implants were stably integrated, innervated, and vascularized up to 2 years. However, the retaining sutures of the implants delayed epithelial closure and there was concurrency of tissue thinning and fibrosis (8). In addition, engineered tissue sheets, thermoresponsive polymeric substrates also used as scaffold structure, have been clinically evaluated using autologous oral mucosal epithelium. However, major drawbacks of this technique are the high variability and the extended time in culture required to generate adequate structures for transplantation (9). Similarly, human amniotic membranes have been extensively applied as substrates for corneal epithelial-derived in vitro expansion and for reconstruction of damaged cornea in several animal models (e.g., rat, rabbit, and goat) (10,11). However, the high inter- and intra-tissue variability in morphological, chemical, and optical properties limit the use of the human amniotic membrane in clinical settings (12,13). Alternatively, human donor corneal stromal tissues have been proposed as substrates for human corneal epithelium growth, displaying, in vitro, features similar to the native limbal epithelium (14). The lack of corneal tissue donor availability significantly affects its clinical potential for corneal reconstruction.
Recently, several groups have succeeded in preparing an acellular corneal stroma by using non-ionic detergents and/or enzymes. Compared to collagen, amniotic membranes or other synthetic materials, acellular corneal stroma has advantage as a potential alternative scaffold structure to transplantation. As early as 2003, Amano et al. suggested that heterogeneous corneal stromas, especially porcine corneal stromas, could be ideal alternatives to human corneas due to their lower antigenicities, as demonstrated in in vitro corneal tissue engineering, and transparently healing, as shown in animal experiments (15). In addition, studies regarding the successful construction of tissue-engineered hearts, lungs, and livers using acellular biomaterials have also been reported (16-18), which indicates that the acellular stroma is a suitable carrier for tissue engineering. Acellular porcine corneal stroma (APCS) has a cell affinity and can provide a relative healthy corneal stroma microenvironment as well as a substrate—the basement membrane that is conducive to corneal epithelial cell adhesion and growth. The mesh structure in the matrix layer can provide space for the growth of mesenchymal cells and the exchange of metabolites. In addition, APCSs can also be used as seed carriers (19). Previous animal study (20), through implantation of APCSs into rabbit corneal pockets and mouse subcutaneous tissue, showed that the APCS is unable to stimulate a host response in the cornea. In subcutaneous tissue, the APCS only triggered an innate immune reaction, which had decreased by 28 days post-implantation. These results demonstrated that complete removal of the cell components of the cornea, which are thought to be the main source of the major histocompatibility complex antigens responsible for allograft/xenograft rejection, can obviously alleviate the immune response to the grafts. In addition, APCS implants could be integrated with body tissues and sufficiently support new tissue regeneration and reconstruction (21). Although many studies in vitro and in animals have confirmed that APCS has excellent potential for solving the problem created by the serious lack of donor corneas, no large-scale clinical study of ACPS has ever been reported. For the first time, our group has proven that APCS is safe and effective in clinical application (22). Through more than 3 years clinical observations, our studies have shown that, similar to human donor corneas, the transplanted areas generally require 3 days for epithelialization in which the epithelial regeneration is the most important factor for reducing postoperative complications. Compared with the biosynthetic cornea reported by Dr. Fagerholm et al. in 2010 (8), which showed that the highest corrected visual acuity of 0.4 was only achieved in six patients with markedly increased post-surgical astigmatism due to degradation of the biomaterials and delayed full epithelialization (for at least 1 month), APCS is far superior because it is similar to the natural cornea on the elastic modulus, has good toleration of sutures, provides cutting tension and permits enzymatic degradation in vivo. All these proved that APCS is a suitable scaffold structure for bioengineering cornea construction.
Seed cells of bioengineering cornea
Another key factor in the construction of tissue engineering cornea is seed cells. The cornea is an organ comprised of three distinct cellular layers, corneal epithelium, stroma, and endothelium, separated by two acellular collagenous interfaces referred to as Bowman’s layer and Descemet’s membranes. Epithelial and endothelial layers play pivotal roles in maintaining corneal deturgescence (relative state of hydration), and ultimately its transparency, through barrier and pump functions (6). However, unlike the epithelium and stroma cells, human cornea endothelium cells (HCECs) are notorious for having a limited proliferative capacity in vivo because of the mitotic block at the G1 phase of the cell cycle due to “contact-inhibition” (23). A considerable research effort has been put into developing alternative methods for construction of tissue-engineered corneal endothelial grafts (Table 1). To circumvent the mitotic block governed by contact inhibition, the conventional engineering method is to disrupt cell-cell junctions with ethylene diamine tetraacetic acid (EDTA)-trypsin to generate single HCECs and then culture them in a medium supplemented with growth factors including basic fibroblast growth factor (bFGF) (33). Unfortunately, this method runs the risk of losing the normal phenotype to endothelial mesenchymal transition (EMT) (34). It has reported that one mechanism leading to EMT is caused by the activation of canonical Wingless-related integration site (Wnt) and TGF-β signaling (34). To mitigate this shortcoming, we collaborated with Dr. Tseng’s group have discovered a novel strategy to unlock the mitotic block in HCEC monolayers, without disrupting cell-cell junctions, by knockdown of p120 catenin (p120) to activate p120-Ras homologue gene family A (RhoA)-Rho-associated protein kinase (ROCK) signaling while sparing canonical Wnt signaling (34-36). To circumvent such a mishap that usually ensues when single HCEC are isolated based on EDTA or EDTA plus bFGF, we digested stripped Descemet’s membrane with collagenase alone (Figure 1) in a serum-containing medium termed supplemented hormonal epithelial medium (SHEM) which consists of DMEM/F-12 (1:1) supplemented with 5% FBS, 0.5% dimethylsulfoxide, 2 ng/mL hEGF, 5 mg/mL insulin, 5 mg/mL transferrin, 5 ng/mL selenium, 0.5 mg/mL hydrocortisone, and 1 nM cholera toxin (36). Because collagenase digestion selectively removes interstitial but not basement membrane collagens, our method does not disrupt intercellular junctions or interaction with the basement membrane. Indeed, collagenase digestion results in compact aggregates of HCEC, which retain intercellular junctions mediated by ZO-1 and connexin-43, and maintain their adhesion to such basement membrane components as collagen IV, laminin 5, and perlecan (35). Presumably because of the preservation of intercellular junctions and cell adhesion to the basement membrane, isolated HCEC aggregates remain viable in a serum-free high calcium medium for at least 3 weeks (37). The aggregate forms a monolayer of hexagonal HCEC expressing ZO-1 and connexin 43 after being re-seeded in SHEM on collagen IV-coated plastic (37). The resultant HCEC monolayer maintains hexagonal shape, expresses all adherent junction (AJ) components, and adopts mitotic block due to contact inhibition after 3 weeks of culturing in SHEM (38). Because we believe that peripheral corneas contain HCEC progenitors and we have an extensive experience with the expansion of limbal niche progenitors in a serum-free medium termed MESCM (35), we thus switched the medium from SHEM to MESCM and interestingly noted dramatic expansion of HCEC monolayers from the size 1.4±0.2 mm of HCEC monolayers in diameter in SHEM to 4.4±0.3 mm in diameter in MESCM after 6 weeks of culture from Descemet’s membrane stripped from 1/8 of the corneoscleral rim normally discarded after conventional corneal transplantation (35). This beneficial effect is mainly due to the delay of contact inhibition by LIF-JAK-STAT3 signaling in the absence of p120-Kaiso knockdown (36). Nonetheless, additional p120-Kaiso knockdown for 5 weeks results in further expansion of HCEC monolayers to 11.0±0.6 mm in diameter (35). This success is achieved by reprogramming adult HCECs into neural crest-like progenitors via activation RhoA-ROCK-canonical bone morphogenetic protein (BMP) signaling that links to the activation of the miR302b-Oct4-Sox2-Nanog network (35). These achievements provide a promising proposal for corneal endothelial seed cells in tissue engineering corneal construction. Successful commercialization of this technology will also stimulate the scientific community to rethink how “contact inhibition” can be safely perturbed to our benefit without pathological consequences and whether this new regenerative approach can circumvent the need of using embryonic stem cells or reprogramming somatic cells all the way to induced pluripotent stem cells.
Table 1
Cell source | Biomaterial | Clinical status | Reference |
---|---|---|---|
Human and rabbit corneal endothelial cells | Silk fibroin films |
|
Vázquez |
Human corneal endothelial cell line HCEC-B4G12 | Decellularization of human corneal stromal lamellae |
|
He |
Human corneal endothelial cells | Human bone derived collagen |
|
Vázquez |
Bovine and human corneal endothelial cells | Engineered basement membranes |
|
Palchesko |
Bovine corneal endothelial cells | Chitosan-polycaprolactone blends |
|
Wang |
Sheep corneal endothelial cells | Ultrathin chitosan-polyethylene glycol hydrogel films |
|
Ozcelik |
Human corneal endothelial cells | Thermo-responsive cell culture carrier |
|
Teichmann |
Human corneal endothelial cells | Denuded amniotic membranes |
|
Fan |
Human corneal endothelial cells | Atelocollagen sheet |
|
Zhu |
HCEC, human cornea endothelium cells.
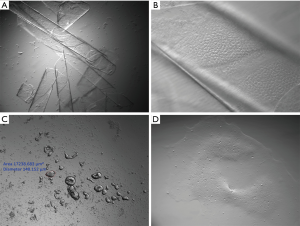
In an effort to address the need for viable human corneas, significant advances in tissue engineering have been made in recent years. Both synthetic and naturally derived biomaterials in combination with primary cells have been used to regenerate and replace partial or full-thickness pathological corneas, starting from epithelial, stromal, endothelial layers, and full-thickness corneal tissues. Among all these scaffold structures, APCS hold promise for long-term success having reached human clinical trials and already get approved (22). The next step’s effort should be made to combine the advantage of APCS and the progress achieved in corneal endothelia researches through collaborative studies, and to allow the study of cornea diseases in vitro as discovery tools for new treatment options.
Acknowledgments
Funding: This work was supported by National Natural Science Foundation of China (Grants: 81600708).
Footnote
Provenance and Peer Review: This article was commissioned by the Guest Editors (Weiyun Shi and Jin Yuan) for the series “Bioengineering Cornea” published in Annals of Eye Science. The article has undergone external peer review.
Conflicts of Interest: Both authors have completed the ICMJE uniform disclosure form (available at http://dx.doi.org/10.21037/aes.2017.12.04). The series “Bioengineering Cornea” was commissioned by the editorial office without any funding or sponsorship. XL reports grants from National Natural Science Foundation of China, during the conduct of the study. MZ reports grants from National Natural Science Foundation of China, during the conduct of the study. The authors have no other conflicts of interest to declare.
Ethical Statement: The authors are accountable for all aspects of the work in ensuring that questions related to the accuracy or integrity of any part of the work are appropriately investigated and resolved.
Open Access Statement: This is an Open Access article distributed in accordance with the Creative Commons Attribution-NonCommercial-NoDerivs 4.0 International License (CC BY-NC-ND 4.0), which permits the non-commercial replication and distribution of the article with the strict proviso that no changes or edits are made and the original work is properly cited (including links to both the formal publication through the relevant DOI and the license). See: https://creativecommons.org/licenses/by-nc-nd/4.0/.
References
- Whitcher JP, Srinivasan M, Upadhyay MP. Corneal blindness: a global perspective. Bull World Health Organ 2001;79:214-21. [PubMed]
- Tan DT, Dart JK, Holland EJ, et al. Corneal transplantation. Lancet 2012;379:1749-61. [Crossref] [PubMed]
- Tandon R, Singh A, Gupta N, et al. Upgradation and modernization of eye banking services: Integrating tradition with innovative policies and current best practices. Indian J Ophthalmol 2017;65:109-15. [Crossref] [PubMed]
- Gain P, Jullienne R, He Z, et al. Global Survey of Corneal Transplantation and Eye Banking. JAMA Ophthalmol 2016;134:167-73. [Crossref] [PubMed]
- Griffith M, Alarcon EI, Brunette I. Regenerative approaches for the cornea. J Intern Med 2016;280:276-86. [Crossref] [PubMed]
- Ruberti JW, Roy AS, Roberts CJ. Corneal biomechanics and biomaterials. Annu Rev Biomed Eng 2011;13:269-95. [Crossref] [PubMed]
- Parker JS, Morris RE, Rooney DM. Boston Type 1 Keratoprosthesis: Visual Outcomes, Device Retention, and Complications. Cornea 2017;36:e13-4. [Crossref] [PubMed]
- Fagerholm P, Lagali NS, Merrett K, et al. A biosynthetic alternative to human donor tissue for inducing corneal regeneration: 24-month follow-up of a phase 1 clinical study. Sci Transl Med 2010;2:46ra61 [Crossref] [PubMed]
- Madathil BK, Kumar PR, Kumary TV. N-isopropylacrylamide-co-glycidylmethacrylate as a thermoresponsive substrate for corneal endothelial cell sheet engineering. Biomed Res Int 2014;2014:450672
- Zhang L, Zou D, Li S, et al. An Ultra-thin Amniotic Membrane as Carrier in Corneal Epithelium Tissue-Engineering. Sci Rep 2016;6:21021. [Crossref] [PubMed]
- Zhao Y, Ma L. Systematic review and meta-analysis on transplantation of ex vivo cultivated limbal epithelial stem cell on amniotic membrane in limbal stem cell deficiency. Cornea 2015;34:592-600. [Crossref] [PubMed]
- Wang HY, Wei RH, Zhao SZ. Evaluation of corneal cell growth on tissue engineering materials as artificial cornea scaffolds. Int J Ophthalmol 2013;6:873-8. [PubMed]
- Connon CJ, Doutch J, Chen B, et al. The variation in transparency of amniotic membrane used in ocular surface regeneration. Br J Ophthalmol 2010;94:1057-61. [Crossref] [PubMed]
- Lin J, Yoon KC, Zhang L, et al. A native-like corneal construct using donor corneal stroma for tissue engineering. PLoS One 2012;7:e49571 [Crossref] [PubMed]
- Amano S, Shimomura N, Kaji Y, et al. Antigenicity of porcine cornea as xenograft. Curr Eye Res 2003;26:313-8. [Crossref] [PubMed]
- Ott HC, Matthiesen TS, Goh SK, et al. Perfusion-decellularized matrix: using nature's platform to engineer a bioartificial heart. Nat Med 2008;14:213-21. [Crossref] [PubMed]
- Petersen TH, Calle EA, Zhao L, et al. Tissue-engineered lungs for in vivo implantation. Science 2010;329:538-41. [Crossref] [PubMed]
- Shupe T, Williams M, Brown A, et al. Method for the decellularization of intact rat liver. Organogenesis 2010;6:134-6. [Crossref] [PubMed]
- Zhu J, Zhang K, Sun Y, et al. Reconstruction of functional ocular surface by acellular porcine cornea matrix scaffold and limbal stem cells derived from human embryonic stem cells. Tissue Eng Part A 2013;19:2412-25. [Crossref] [PubMed]
- Luo H, Lu Y, Wu T, et al. Construction of tissue-engineered cornea composed of amniotic epithelial cells and acellular porcine cornea for treating corneal alkali burn. Biomaterials 2013;34:6748-59. [Crossref] [PubMed]
- Du L, Wu X. Development and characterization of a full-thickness acellular porcine cornea matrix for tissue engineering. Artif Organs 2011;35:691-705. [Crossref] [PubMed]
- Zhang MC, Liu X, Jin Y, et al. Lamellar keratoplasty treatment of fungal corneal ulcers with acellular porcine corneal stroma. Am J Transplant 2015;15:1068-75. [Crossref] [PubMed]
- Joyce NC. Cell cycle status in human corneal endothelium. Exp Eye Res 2005;81:629-38. [Crossref] [PubMed]
- Vázquez N, Rodríguez-Barrientos CA, Aznar-Cervantes SD, et al. Silk Fibroin Films for Corneal Endothelial Regeneration: Transplant in a Rabbit Descemet Membrane Endothelial Keratoplasty. Invest Ophthalmol Vis Sci 2017;58:3357-65. [Crossref] [PubMed]
- He Z, Forest F, Bernard A, et al. Cutting and Decellularization of Multiple Corneal Stromal Lamellae for the Bioengineering of Endothelial Grafts. Invest Ophthalmol Vis Sci 2016;57:6639-51. [Crossref] [PubMed]
- Vázquez NN, Chacon M, Rodríguez-Barrientos CA, et al. Human Bone Derived Collagen for the Development of an Artificial Corneal Endothelial Graft. In Vivo Results in a Rabbit Model. PLoS One 2016;11:e0167578 [Crossref] [PubMed]
- Palchesko RN, Funderburgh JL, Feinberg AW. Engineered Basement Membranes for Regenerating the Corneal Endothelium. Adv Healthc Mater 2016;5:2942-50. [Crossref] [PubMed]
- Wang TJ, Wang IJ, Lu JN, et al. Novel chitosan-polycaprolactone blends as potential scaffold and carrier for corneal endothelial transplantation. Mol Vis 2012;18:255-64. [PubMed]
- Ozcelik B, Brown KD, Blencowe A, et al. Ultrathin chitosan-poly(ethylene glycol) hydrogel films for corneal tissue engineering. Acta Biomater 2013;9:6594-605. [Crossref] [PubMed]
- Teichmann J, Valtink M, Gramm S, et al. Human corneal endothelial cell sheets for transplantation: thermo-responsive cell culture carriers to meet cell-specific requirements. Acta Biomater 2013;9:5031-9. [Crossref] [PubMed]
- Fan T, Ma X, Zhao J, et al. Transplantation of tissue-engineered human corneal endothelium in cat models. Mol Vis 2013;19:400-7. [PubMed]
- Zhu YT, Tighe S, Chen SL, et al. Engineering of Human Corneal Endothelial Grafts. Curr Ophthalmol Rep 2015;3:207-17. [Crossref] [PubMed]
- Hatou S, Yoshida S, Higa K, et al. Functional corneal endothelium derived from corneal stroma stem cells of neural crest origin by retinoic acid and Wnt/beta-catenin signaling. Stem Cells Dev 2013;22:828-39. [Crossref] [PubMed]
- Zhu YT, Chen HC, Chen SY, et al. Nuclear p120 catenin unlocks mitotic block of contact-inhibited human corneal endothelial monolayers without disrupting adherent junctions. J Cell Sci 2012;125:3636-48. [Crossref] [PubMed]
- Zhu YT, Li F, Han B, et al. Activation of RhoA-ROCK-BMP signaling reprograms adult human corneal endothelial cells. J Cell Biol 2014;206:799-811. [Crossref] [PubMed]
- Liu X, Tseng SC, Zhang MC, et al. LIF-JAK1-STAT3 signaling delays contact inhibition of human corneal endothelial cells. Cell Cycle 2015;14:1197-206. [Crossref] [PubMed]
- Li W, Sabater AL, Chen YT, et al. A novel method of isolation, preservation, and expansion of human corneal endothelial cells. Invest Ophthalmol Vis Sci 2007;48:614-20. [Crossref] [PubMed]
- Zhu YT, Hayashida Y, Kheirkhah A, et al. Characterization and comparison of intercellular adherent junctions expressed by human corneal endothelial cells in vivo and in vitro. Invest Ophthalmol Vis Sci 2008;49:3879-86. [Crossref] [PubMed]
Cite this article as: Liu X, Zhang MC. The present and the prospect of bioengineering cornea. Ann Eye Sci 2018;3:7.